The Early Earth and Life’s Origins
- ScienceDuuude
- Aug 31, 2020
- 12 min read
“…In the beginning God created the heaven and the earth. And the earth was without form, and void; and darkness was upon the face of the deep...” – Genesis 1:1
“…whilst this planet has gone cycling on according to the fixed law of gravity, from so simple a beginning endless forms most beautiful and most wonderful have been, and are being, evolved.” – C. Darwin
“…Ummm, hello… could someone turn on the light…” – S. Duuude
1. Earth without form…
In both human and planetary births, the parent’s context is often critical to the health or even existence of the offspring. For example, if our sun was born in a stellar nursery with a supergiant star, fierce stellar winds from such a big hot star would have driven away the dust and gas, the raw materials of planet-formation surrounding our infant sun. Instead, our small, calm sun was born in a quiet corner of the galaxy, and the Earth was born along with its protective siblings in the solar system. The Earth, in its turn, produced Life. We’ll explore some of the early Earth’s context and how that affected the origin of life.
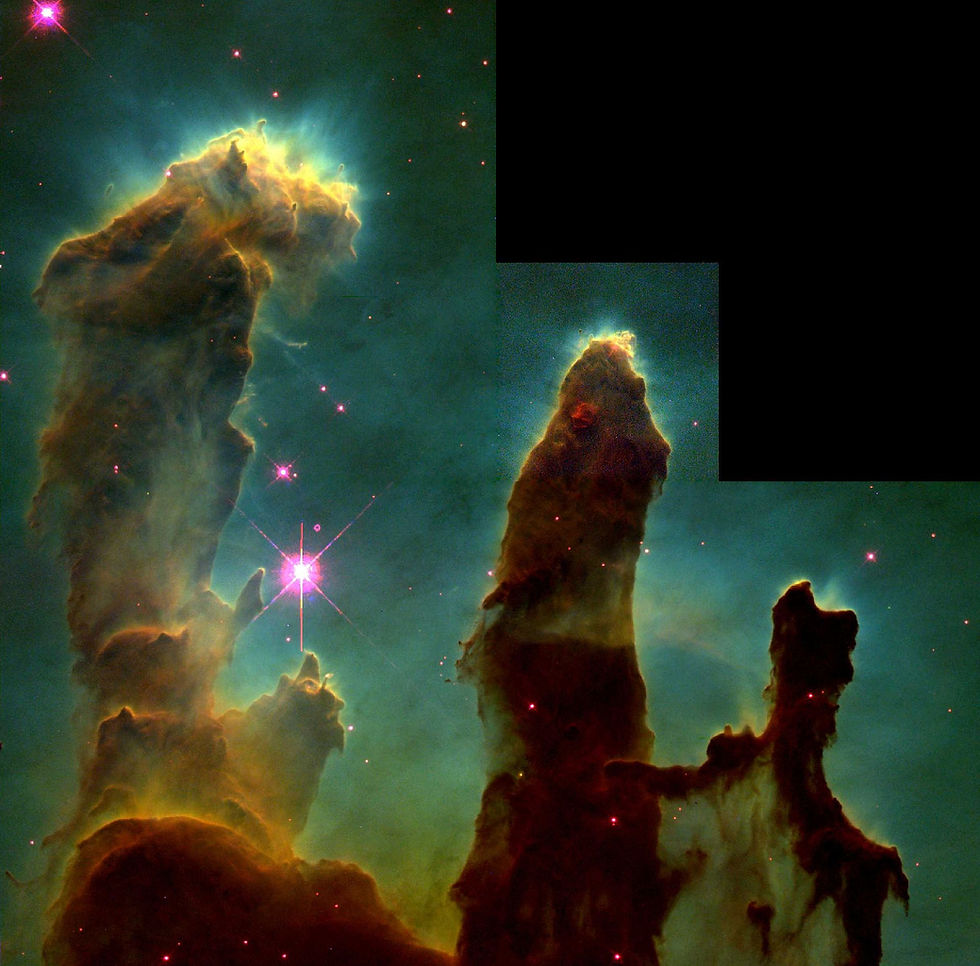
The sun first ignited about 4.65 billion years ago and quickly burned away most of the gas and dust, the elemental products of stellar furnaces, leaving a compact protoplanetary disk around it. The first solar system solids condensed quickly from that disk about 4.57 billion years ago and then rapidly evolved into a bumper car ride of planetesimals (asteroids between a 1-1000 km) and protoplanets (greater than 1000 km), crashing into each other. The big ones grew bigger fastest by gravity, quickly clearing out the disk, and soon the inner solar system of terrestrial planets developed: Mercury, Venus, Earth, and Mars.
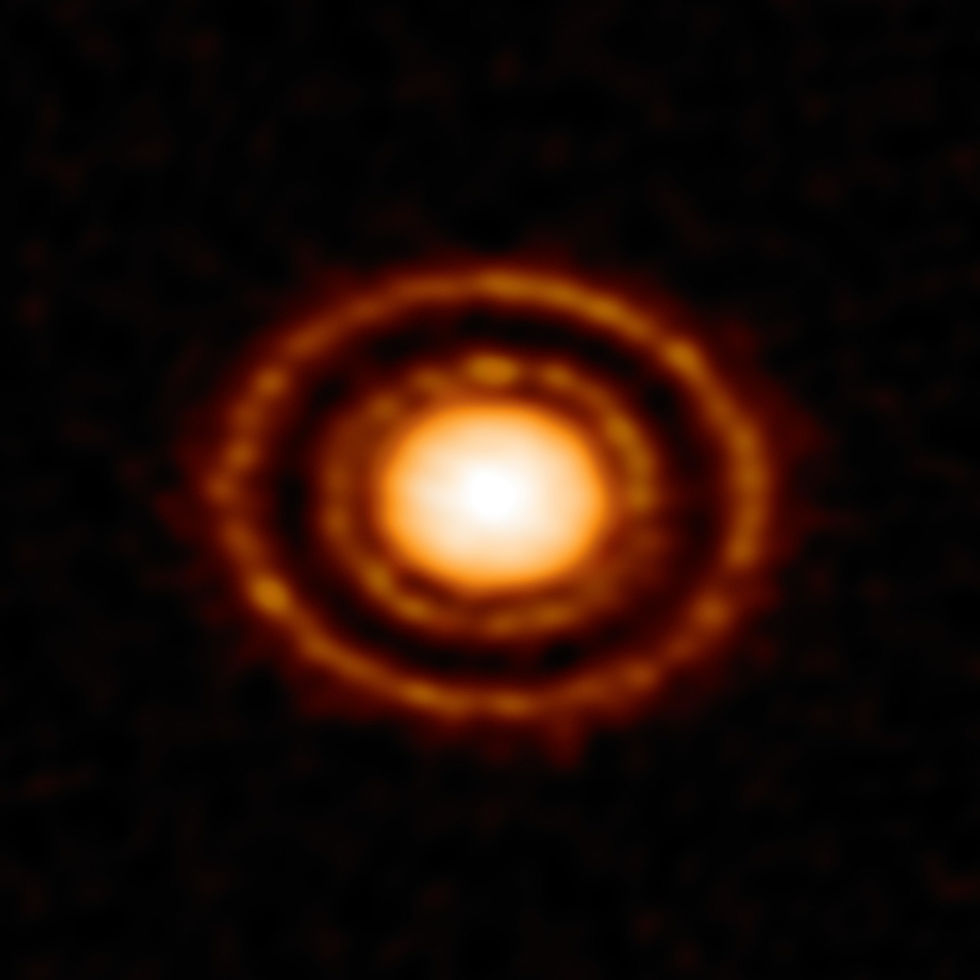
About 4.56 billion years ago, still in the early phases of planetary development, a Mars-sized protoplanet crashed into the young Earth and vaporized much of the surface materials in both. The vapor cloud continued to orbit around the molten core of Earth, and eventually coalesced into two bodies, the Moon and Earth, each with identical surface chemistries, and matching orbital and rotational periods. There was no water on these two bodies at this time, since the asteroids that formed them were baked dry by their proximity to the sun.
The Earth and Moon remained molten for about 30 million years before continental crusts finally solidified. Then, Jupiter and Saturn migrated first in towards the sun, then back out, thus disrupting the orbits of asteroids in their vicinity. Those asteroids were farther away from the sun, and colder, and unlike the ones closer to the sun, were rich in water and organic materials. As those cold asteroid’s orbits degraded, they plunged towards the sun and some crash-landed those life-essential materials into the rocky planets including Earth. This was the Late Heavy Bombardment, around 4.4 billion years ago. The size and frequency of huge asteroid impacts during this bombardment occasionally vaporized the Earth’s developing oceans. It took time once the heaviest of the collisions began to taper off for the oceans to reform and cool to near boiling temperatures.
Zircon chemistry suggests that Earth had a crust with liquid water by about 4.3 billion years ago. There are no rocks from that time or before to bear witness, as plate tectonics began about then and eventually erased almost all evidence. Zircons, tiny indestructible crystals of zirconium and silicon oxides that form as magma cools, are all that remain of those ancient rocks. But zircons are little time capsules that entrap within them hints of the environment in which they were born.
2. Gathering together of the waters…
The addition of water to the dry molten Earth during the Late Heavy Bombardment was the seed for many important developments in the geology and life of our planet, not just the obvious formation of oceans and other surface waters. For example, the addition of water to solidifying magmas is required for continental crust to form. Water reacts with the lithosphere (the upper solid part of the earth) to weaken it and initiate plate tectonics, where it also helps to lubricate the plates as they subduct (dive hundreds of kilometers down into the mantle). Water also helps rock to melt which is the key to volcanism near subduction zones. In fact, water has powerful effects on almost all aspects of geology from the chemistry of the rocks, to their melt temperatures, to mechanical properties, etc.
Where the ocean enters the Earth through faults, cracks in the crust, water penetrates far into the mantle and reacts with the hot silicate rocks there and leaches out minerals, metals, and gases. The superheated chemical-laden water eventually works its way out through seafloor hydrothermal vents.
Hydrothermal vent’s rich chemistry, tremendous chemical and thermal energy, and plentiful catalytic surfaces make them ideal for synthesizing the various biochemicals necessary for life. The extreme depths and hadean blackness of hydrothermal vents provide both challenges and advantages for the origin of life. The lack of sunlight, of UV radiation as an energy source, is a challenge to some theories of how life began. The lack of tides and a wet-dry cycle from the young moon similarly challenge some ideas of the cradle of life. But the kilometers of water above the vent also provided a buffer from the continued pummeling of asteroids and other assaults that may have occasionally sterilized early attempts at life near the surface.
Along with the hot new oceans was a hot early atmosphere which was dominated by molecular nitrogen (N2), carbon dioxide (CO2), and smaller amounts of hydrogen (H2). Water vapor (H2O) was also a large part of this early atmosphere. This was not the easiest composition of gases with which to create the chemicals of life.
If, instead, a reducing atmosphere (of highly reactive gases) had developed as imagined by the early origin-of-life theorists and experimenters, an atmosphere heavy in methane, ammonia, hydrogen, it would have been easier for organic chemistry. But it would not have lasted long. The hydrogen would have escaped into space after reacting with ultraviolet radiation pouring in from the sun and breaking down the ammonia and methane. No one’s view of the early atmosphere had oxygen present at that time.
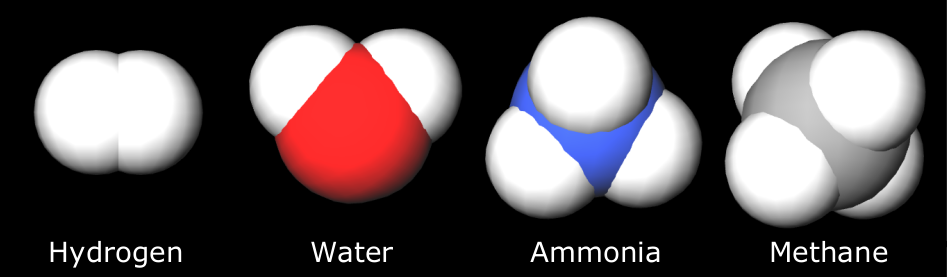
However, there was an overabundance of productive high energy sources in the atmosphere, from lightning to ultraviolet radiation, traumatizing the molecules of gas to synthesize biochemicals. This synthesis happened in the atmosphere and even in clear water where UV energy penetrated to depths of about 10 meters.

Hot springs on land, near volcanos, were another source of life’s chemicals. Ponds that can flood with hot chemical-rich waters one day, and dry out the next, drove a cycle of wet-dry chemical reactions needed to synthesize simple and complex biochemicals. A wet-dry cycle features in many theories of prebiotic synthesis, and here a hyperactive moon is often conjured. Some early models of moon-formation had the moon closer to the Earth causing extreme tides every six hours. The moon would have hovered over toxic skies appearing twice the size of today since its orbit was half the radial distance.
In addition to terrestrial chemistry, asteroids from the neighborhood of the gas giants delivered compounds essential to life onto the young Earth. Water. Amino acids. Nucleobases. Sugars like ribose. Fatty acids. And more.
These are just some of the many ways that organic compounds essential for life accumulated on the early Earth.
The ubiquity of water and of basic biochemicals throughout the universe, and particularly on various bodies throughout our solar system, suggests that the chemistry needed for life’s precursors is not rare. In fact, some researchers propose that the early Earth was covered in a layer of organic compounds, a scum of oily residue that coated the water and the land. With perhaps a haze of organics polluting the air above. The question is not so much that there were organic compounds on the early Earth, but what specific conditions led to the abundance of those chemicals needed for life to arise.
John D. Sutherland, a chemist at the Medical Research Council Laboratory of Molecular Biology in Cambridge, England, may have gotten the furthest so far in cobbling together a plausible set of reactions necessary for life to begin. In 2015 his lab was able to show that a common set of chemical steps could drive the formation of all the important biological molecules (ribonucleotides, amino acids and lipids) from simple precursor chemicals such as hydrogen cyanide, powered by UV light.
The opening line of his February, 2020 paper in Science Advances is probably at least as important as a strategy and call to action, as the technical information he reviews in that paper:
“We advocate an integrative approach between laboratory experiments in prebiotic chemistry and geologic, geochemical, and astrophysical observations to help assemble a robust chemical pathway to life that can be reproduced in the laboratory.”
3. Let the waters bring forth abundantly…
So, imagine we had an ocean saturated with the raw materials of life, the primordial soup. How did the raw materials self-assemble into the first protocell? Or say we had a hot spring, a pond, something shallow on land that could cycle between wet and dry - how did we get from a warm shallow pool of organic compounds to life? Or we had a black smoker hydrothermal vent at the bottom of a primeval ocean, spewing tons of chemicals and pulsing with every kind of energy except solar - how did that drive the origin of life?
If time were a measure of difficulty, then conjuring life from non-living pond scum was apparently not a difficult step on the planet Earth. From having a hot crust with boiling water about 4.3 billion years ago, we had our first clear signs of bacteria by 3.7 to 3.85 billion years ago. Let’s call it half a billion years for life to become well established enough to leave clear fossils.
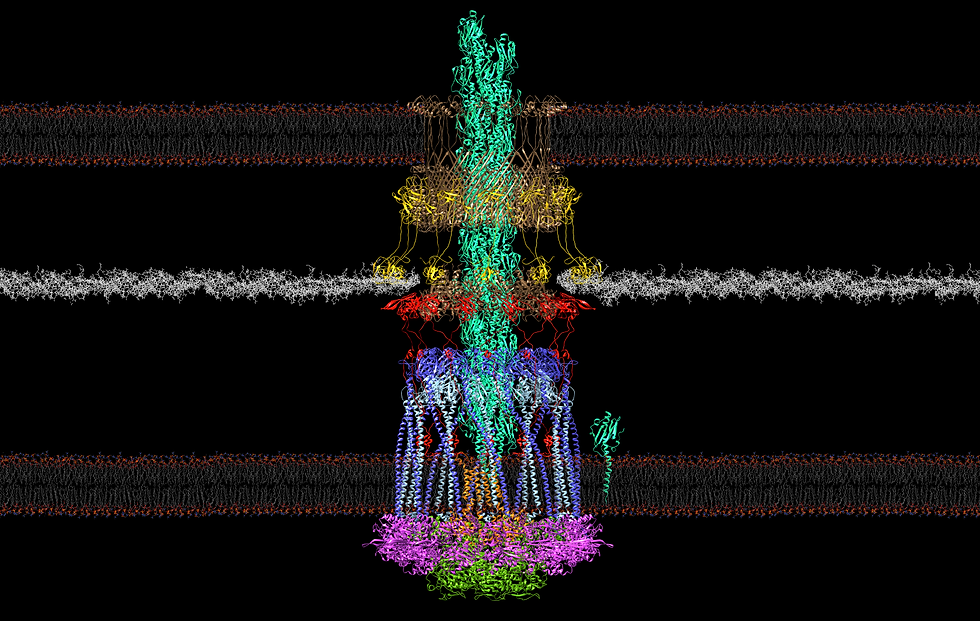
The first evidence of a eukaryote, a cell with a nucleus like we have, was about 1.65 billion years ago. That took more than twice as long, well over a billion years, for prokaryotic (un-nucleated) cells to develop into eukaryotes, than for life to form in the first place.
The first evidence of multicellular life was about 635 million years ago. It took another billion years for single eukaryotic cells to figure out how to work as a team.
It took only half a billion years to evolve from a squishy ball of cells floating in water, to humans typing about that earlier life, gossiping to other humans they don’t know, about how simple and untalented that ball of cells was. Apparently creating humans was not a particularly difficult process either.
How did life arise if it was so easy that it only took half a billion years?
Here is one more perspective on the ease or difficulty of creating life...
One of the central functions of a living thing today is to store, process, and duplicate information - information on how to build itself, and how to function… today DNA and RNA carry that information. We have reason to believe that RNA preceded DNA. How do we get from the building blocks (nucleotides) to a string of RNA carrying the code for our earliest cell? And once we have that RNA, how would the information in it be processed or duplicated?
One of the central functions of a living cell is to catalyze reactions, to extract energy from its surroundings, to synthesize new biochemicals, to read the information in the DNA, make messages and to duplicate that store of information, etc. Today proteins do almost all of that enzymatic work within a cell. If you have all the amino acids in the primordial soup, how do you link them into a functional protein? More importantly, how do you repeat that unlikely act? How do you store the instructions for making that protein?
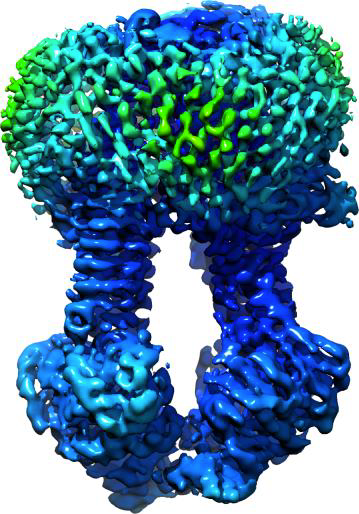
One of the most important and defining parts of a cell is the membrane, because it separates the cell from its environment, and ensures that within the cell are the perfect conditions for all the chemistry of life. Today we have cell membranes composed of lipids which define the boundary of a cell. If you have simple bubble-like membranes, how do you package the right chemicals, and how do the first bubbles grow and divide?
The conundrum appears more intellectual than physical since an abiotic world solved the problem in a relatively short time. How complicated could a bacterium be to make? Let’s tease that apart just a bit.
A bacterium has a few thousand genes that carry instructions for making proteins. Those thousands of genes carry on average a few thousand letters of code for each protein. The millions of letters of genetic code are not the extent of the complexity in a tiny single-celled bacterium. It goes far beyond that.
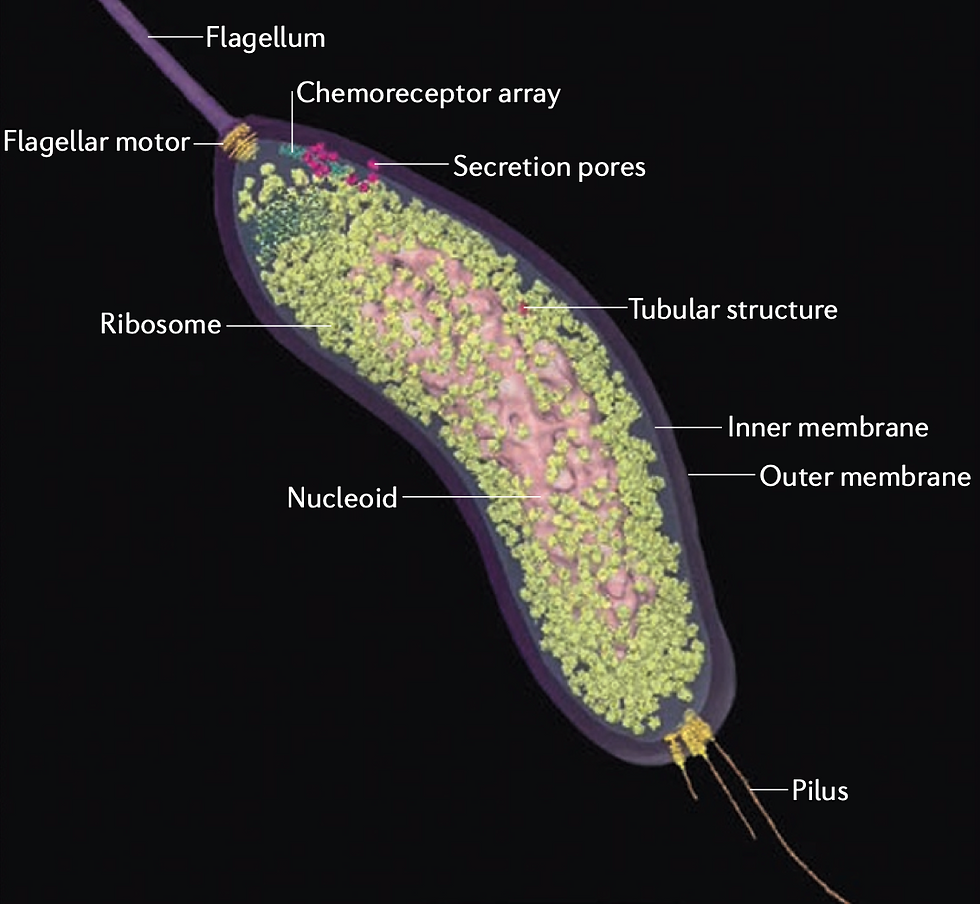
Each protein has a complex three-dimensional shape that the gene encodes, and that shape is essential for its function, which is often as an enzyme to catalyze some specific reaction. Each protein, like our enzyme of interest, not only has to bind to the thing it will catalyze (could be another protein, a nucleic acid like DNA, a fatty acid, sugar, anything really), but our enzyme also binds to other proteins.
Most often those other binding proteins regulate our enzyme of interest, telling it when to turn on and when to turn off. Our enzyme might bind dozens or hundreds of other proteins during its work in the bacterial cell. And each of those regulating proteins may bind to dozens or hundreds of other proteins, and each of those… you get the idea.
The bacterial cell’s proteins form a complex network of interactions. The picture of the total network of interacting proteins in a simple bacterial cell looks like a fuzz ball, and is called an interactome (each point represents a protein, and each line between points represents a binding between those two proteins).

But proteins also interact with every other chemical in the cell. Each nucleic acid, each nutrient and signaling molecule, all those chemicals are also part of an even larger network.
Untangling that fuzz ball to try and reconstruct which biochemicals came first in the earliest protocell is a crazy hard problem.
We are nowhere near cracking the problem, but one of the leading researchers in the field, John W. Szostak at the Massachusetts General Hospital, has a picture starting to form on how this chemical evolution leading to life could have played out.
4. Be fruitful and multiply…
Small non-living bubbles made of fatty acids enclosed a soup with random sequences of some kind of oligonucleotides, chemicals similar to but not exactly like our RNA or DNA. The watery environment supplied most of the biochemicals that were transported across the fatty acid membrane and into these bubbles.
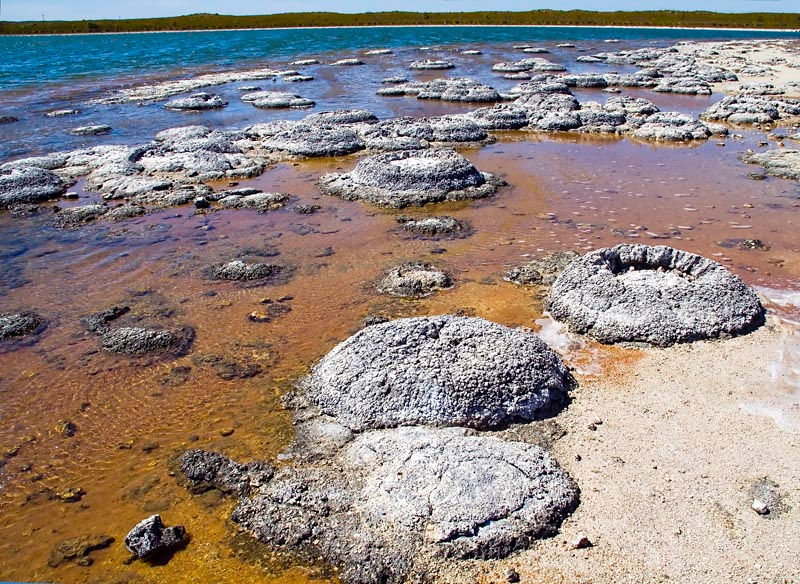
The environment was also critical to the growth and division of these bubbles, since the bubbles had no machinery to control those processes. Newly made bubbles of fatty acids merged with the existing primitive bubble-cells and contributed to their growth. New kinds of lipids such as phospholipids introduced into the film of the bubble enabled it to grow at the expense of bubbles without those novel phospholipids. This might have been one of the earliest examples of pseudo-Darwinian competition.

This simple physical difference of having a tiny bit more phospholipid, and the resulting competitive benefits, drove the selection of bubbles with more phospholipids. Eventually some of these little bubbles had so much phospholipid that it changed the properties of the membrane. It became harder for the bubbles to import biochemicals across a phospholipid-heavy membrane, than across the earlier fatty acid one.
Now there was a new selective pressure – for a bubble to develop a simple membrane transport machine. A small, simple protein, a short chain of amino acids, became a tremendous competitive advantage because it happened to shuttle important biochemicals across the otherwise impermeable phospholipid membrane.
Suddenly there was yet another selective pressure – for a bubble to develop the means to make more proteins to maintain and improve membrane transport. RNA is the enzymatic heart of ribosomes today, the cellular machine that makes proteins. This is unlike almost every other enzyme which are almost always proteins. Ribozymes are much rarer but occur in a number of very core cellular processes like translation of RNA to protein. The ancestral ribozyme at the heart of protein synthesis may have begun here.
Maybe the protein synthesis ribozyme was the first RNA, or maybe not. Maybe it wasn’t even an RNA at first. But somewhere and somehow RNA came into the picture. Once RNA arrived within the cell perhaps with a functional role such as protein synthesis, the ability of RNA to store information arose naturally. This came about as the RNA soon showed an ability to copy itself within the cell.
This is as far as the Szostak paper takes the speculative story of the origin of life. The important thing is that his lab and others have piecemeal, and experimentally, demonstrated some of the steps within that story.
It is not the only story. And the story is neither complete nor convincing as it is told. Yet so far this may be the best story we have on the origin of life.
5. And it was so…
Well, we don’t know if it was so. Almost every point of this story is contested. But scientists seem to be coalescing around the basic outlines of the story: that the solar system is about 4.65 billion years old, the Earth and Moon are about 4.56 billion years old, that the Earth had a crust and water by about 4.3 billion years, and not long after that the prebiotic phase of chemistry began its formative period. Then quite rapidly, somewhere around 4 billion years ago, life had begun. Life was so prevalent and successful that our first fossils of these tiny, fragile, single-celled animalcules were made somewhere around 3.8 billion years ago. We have those rocks, those fossils, and scientists continue to arm-wrestle over whether the oldest ones are evidence of life or not. But at that point, the argument is over a hundred million years here or there. Most seem to be within a rounding error of 4 billion years.
The rest of the story is contested so vigorously it is hard to make a consensus narrative. Were the basic biochemicals like amino acids and nucleotides and fatty acids mostly synthesized on Earth or transported here on the backs of asteroids? Did informational, or metabolic, or membrane components come first? What was the sequence of protocell assembly? How did the protocell work? How did it evolve? How did it step across the threshold into Life? We only know that it did so. And behold, it was very good.
Comments