The Birth of Our Life-Sustaining Solar System
- ScienceDuuude
- Aug 17, 2020
- 17 min read
Updated: Aug 19, 2020
“…We’re made of star stuff…” — Carl Sagan
The stuff of life is forged in stars and dispersed by their deaths…
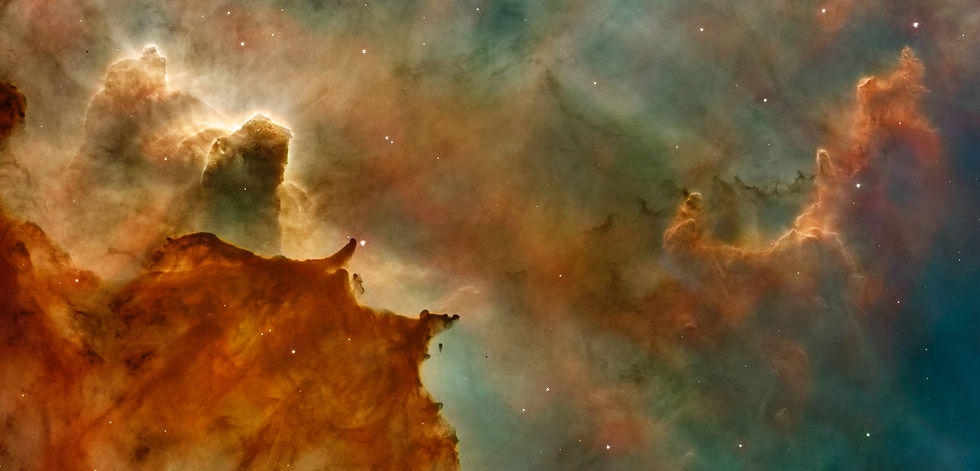
The first elements made within minutes after the Big Bang were mostly hydrogen. About a quarter of the nascent universe’s total mass at the time was helium, and then there was a little lithium. That was it. How did we get from such a simple and monotonous beginning to the complexities of life here on Earth?
The first stars formed within 100 million years after the Big Bang, after things had cooled off enough for gravity to take over and gather the atoms together. Once those stars lit up, we had our first fusion reactions in the cores of stars where the temperatures reached into the millions of degrees Kelvin, hot enough to fuse the nuclei of atoms. Hydrogen fused into helium. Helium made carbon, oxygen, and fluorine… carbon formed magnesium, sodium, neon… oxygen fused into sulfur, phosphorus, silicon… silicon in turn yielded titanium, vanadium, chromium, manganese, iron and more… most of the elements of the periodic table were made within stars, and especially in very large stars.
But stars have limited lifetimes. Their fuel doesn’t last forever. Stars the size of our sun can last ten billion years. Supergiant stars, ten times the mass of our sun, might live only 25 million years. At thirty times our sun’s mass, stars may live only 6 million years. The universe is at least 12.8 billion years old. Plenty of time for many generations of stars, especially the big ones.
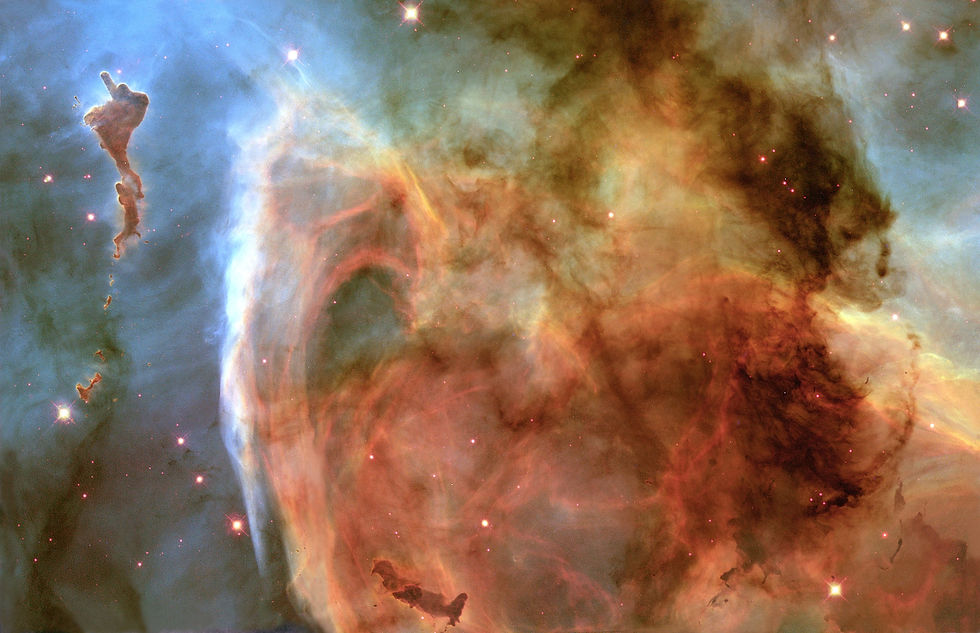
The smaller stars like our sun go quietly into the evening when their time is done. But as they go, they shed their outer layers and disburse the elements forged within their cores throughout their long lives.
The bigger stars, though, make quite a scene (and the scene I describe next is only one of several paths these big stars can follow, and depends on their mass and composition). If a star is at least eight times the mass of our sun, there is a good chance it will go supernova. The key is iron, which has the most tightly bound nucleus of all the elements and does not readily undergo the nuclear fusion process that powers stars. Therefore, as iron accumulates in the core of these supergiant stars, it is like poison accumulating in a body… small amounts may not do anything noticeable, but beyond a certain threshold the poison is lethal.
The threshold for stars is just shy of one and a half times the mass of our sun. That much iron in the core of a supergiant, in combination with depletion of fuel in the upper layers of the star, means that the compressive force of gravity wins the tug-of-war against the expansive pressure of thermonuclear fusion.
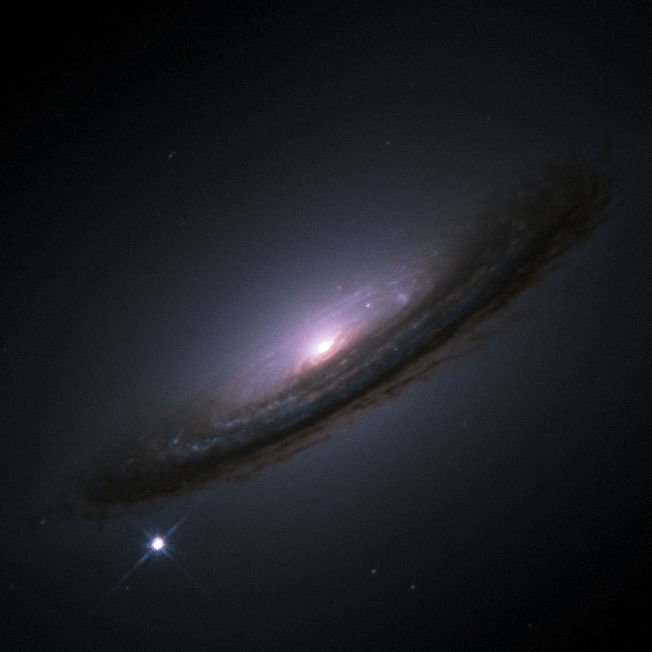
When the old supergiant passes that threshold, gravity squeezes matter so hard, that the electrons get kicked out of their orbital shells and the nuclei of the iron atoms are crushed together. The entire core of iron is crushed this way, one and a half suns worth of iron, squeezed down into a sphere only 20 kilometers across. The average American’s commute is 30 km, or 50% more than the diameter of that core. The iron core of that star has now collapsed into a proto-neutron star. What you have is essentially a gigantic atomic nucleus.
The core’s collapse releases an immense burst of energy which blasts the remaining shells of the supergiant far out into interstellar space. When a star in our own Milky Way galaxy goes supernova its brightness can exceed that of the hundreds of billions of stars in the galaxy, and we might even be able to see it during the day. The visible light of a supernova is caused by radioactive decay in the ejected material, and not by the core collapse itself. But that visible light is a tiny portion of the electromagnetic radiation emitted in a supernova of this type. In turn, the photon radiation is a tiny portion of the energy absorbed by matter, resulting in it being violently thrown into space. And the energy absorbed by matter is a tiny portion of the total energy released by the the core collapse, most all of which goes into releasing a vast torrent of neutrinos (those ghostly particles that rarely interact with matter).
Smaller stars can also go supernova if they are part of a binary system. One way is if they collide. Another way is towards the end of their lives. Old stars too small to undergo core collapse into a neutron star, instead become a red giant and shed their outer layers into a planetary nebula, and the core of the small star becomes a white dwarf. If the white dwarf has a partner star which loses material to the dwarf star, this can also trigger a supernova. Since smaller stars are so much more numerous than supergiant stars, these kinds of supernovas are much more common than the supernovas of massive stars detailed above.

The Crab Nebula is an example of the remnants of a supernova that exploded in the year 1054 AD and was visible during the day for almost a month. After about 900 years the Crab Nebula is about 11 light years across and is expanding at a rate of about 0.5% of the speed of light.
Nebulae like the Crab Nebula are beautiful fleeting objects and last only about ten-thousand years. The hot nebular materials, measuring about 10,000 degrees C in their fluorescent art-work phase, eventually cool down and wink out, and all the gas and dust disperse throughout the region around the neutron star. The materials from the supernova eventually form cold molecular clouds, which can become stellar nurseries birthing clusters of stars with the right trigger.
Debris from the death of stars seed molecular clouds…
Supernovas disperse the elements forged in the hearts of stars. The quieter death of smaller stars results in most of their materials disbursed into planetary nebulas. All that death feeds the next generation of stars and star systems. If stars retained all the materials after their nuclear furnaced burned out, there would be little to build a complex and interesting and living universe like what we see today. Fortunately, stardust is scattered in cold, dark molecular clouds, waiting for the next step.
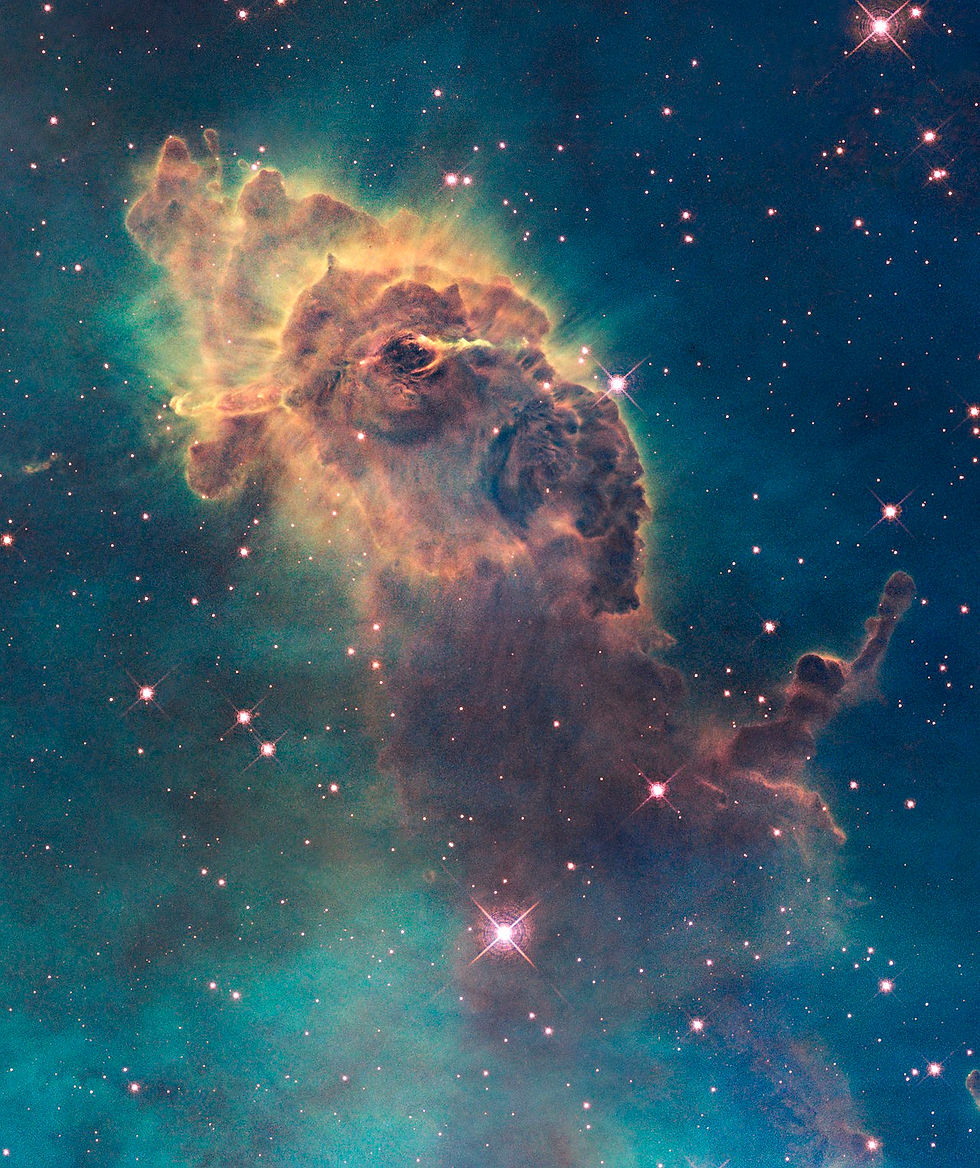
Molecular clouds are composed of mostly hydrogen and helium, like the rest of the universe, but they are also seeded with the dust and debris of heavier elements created in stars of all sizes.
Carbon is one of the critical elements made by stars since it supports the chemistry that enables life. The carbon made in stellar cores is blown out into space, and there it reacts with the other elements that have come along with it: oxygen, nitrogen, sulfur, hydrogen, etc. These elements react to form simple compounds we’ve detected in interstellar space. Compounds like water, carbon dioxide, methane, methanol, ammonia, sodium chloride, etc. And these stellar elements also form complex organic compounds with up to at least a dozen carbons. Glycine, the simplest amino acid, one of the twenty building blocks of proteins, has been detected in these far-flung reaches of space. Laboratory experiments show that interstellar conditions are suitable for synthesis of all the bases needed for DNA and RNA.
Other elements made by stars are just as important for life as those needed for organic compounds. For example, the alkali metals such as sodium and potassium are essential for almost all cellular processes. Transition metals such as iron and copper are necessary for oxygen transport. Other metals like magnesium and manganese are essential cofactors for enzymes to function, and calcium is required for hard tissues like bone and shell. These are just a few obvious examples of the heavy star-made elements necessary for life.
But before we can have life, we need a place for life to thrive. We need a solar system. We need a sun that can host one or more planets where life can begin and carry on.
A given molecular cloud can have enough material to make millions of sun-sized stars and solar systems. We expect gravitational collapse to trigger star formation in these molecular clouds, but instead they often remain puzzlingly inert and stable. This is because various pressures counteract the gravitational forces that would otherwise collapse the cloud material into stars. Shock waves from some external source, like another supernova, are often needed to trigger star formation in molecular clouds.
Wolf-Rayet star triggered a molecular cloud to make our solar system…
About 4.6 billion years ago, a huge and incredibly hot, bright star more than twenty-five times larger than our sun was blasting away its own outer hydrogen shell with its intense stellar winds, eventually leaving only the helium core. This created a wind-bubble around the massive star, with very little material within the bubble. The shock wave, or the shell of the bubble, was dense with the materials blasted away from the star. These huge and highly energetic stars are called Wolf-Rayet stars (WR star) after two 19th century French astronomers who discovered these rare beasts from their Paris observatory.
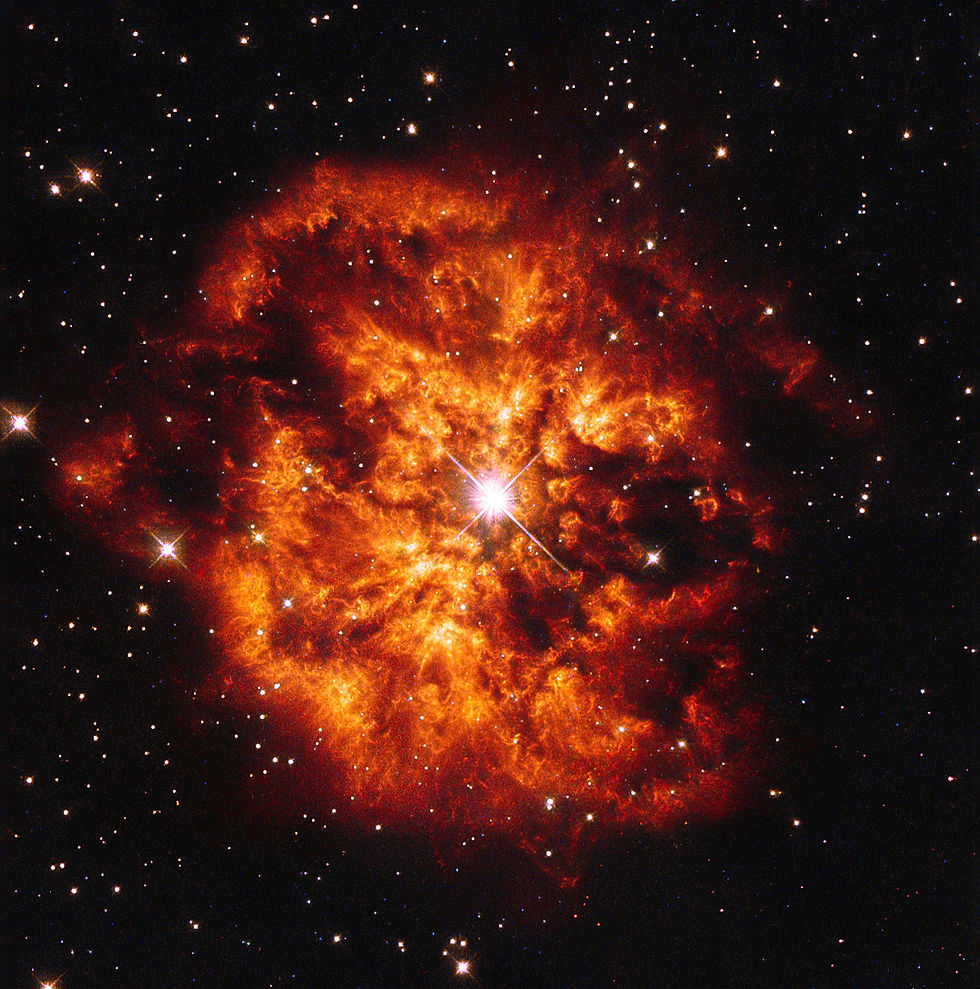
When the WR star’s hot wind-bubble encountered a cold molecular cloud, the shell of the bubble swept up whatever interstellar materials lay in its path, like a line of debris pushed ahead of a broom. The violent collision caused these materials to glow in various wavelengths. The concentration of materials swept up by the shock waves of the wind-bubble triggered a multitude of star births. We see such stellar nurseries at the boundaries of WR star wind-bubbles today.
That 4.6 billion year old WR bubble triggered the formation of our own solar system. Hot giants like WR stars and other supergiants do not live long, so we can’t point at a star and wave happily to our stellar midwife. So, where’s the evidence then?
The key evidence was found by a team from the University of Chicago and Clemson University, in the ratio of aluminum and iron isotopes. In our solar system, the ratio of aluminum isotopes (of atomic mass 26 to 27) is about 17 times higher than in our Milky Way galaxy. Meanwhile, the ratio of iron isotopes (atomic mass 60 to 56) is lower than the galactic average.
Al-26 is made in the outer layers of stars and is one of the byproducts of hydrogen fusion (where a proton is captured by Mg-25). The radioactive Al-26 is ejected in the solar winds of WR stars and deposited deep into surrounding molecular clouds, like a fishing boat carried miles inland by a tsunami. Fe-60 is made in the core of stars, and the only way it is expelled out into space is by a supernova explosion.
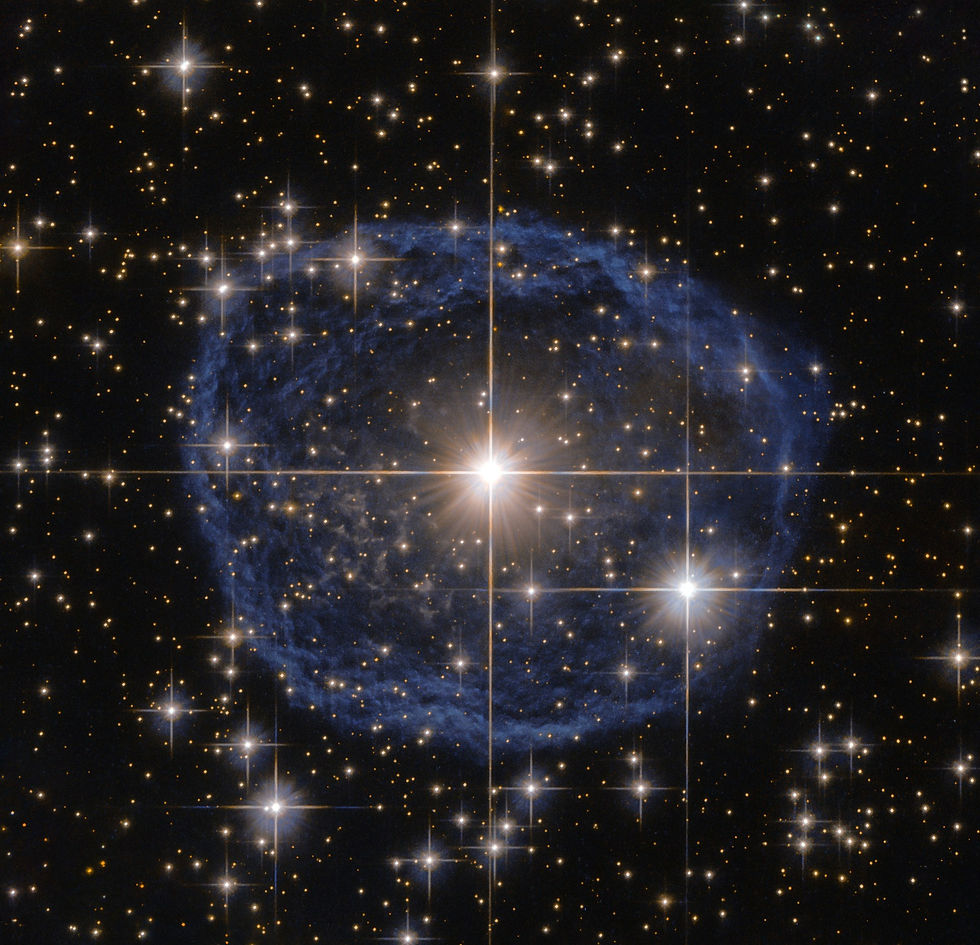
The best explanation, therefore, for such a high ratio of Al-26/Al-27 and low Fe-60/Fe-56 compared to the galactic average is that dust and debris from a WR star’s wind-bubble, and not a supernova, triggered the formation of our solar system.
There’s an interesting side note about Harold Urey, the Ph.D. advisor of Stanley Lloyd Miller, who’s experiment ushered in a new era of origin of life studies, and who we wrote about here. Urey hypothesized in a 1955 paper that Al-26 was the radioactive isotope responsible for heating and melting the core of the Earth and other rocky planets in our solar system, rather than radioactivity from isotopes of potassium, uranium, and thorium. Urey also speculated that Al-26 was formed in stellar processes.
To put Urey’s paper into perspective we need to introduce Fred Hoyle, the man who founded the field of nucleosynthesis, the study of how stars make elements from hydrogen. Fred Hoyle was an English astronomer who calculated in a remarkable 1946 paper that the temperatures within a collapsed star are high enough to synthesize the elements of the periodic table from hydrogen. He followed this up in a 1955 paper which focused on the synthesis of elements between carbon and nickel in concentric shells of a supergiant star. But it was not until 1957 that Hoyle and a team of other astrophysicists published the foundational thesis of supernova nucleosynthesis which set the standards for the field for the rest of the century. The 1957 paper eclipsed Hoyle’s earlier paper despite Hoyle being senior author on the later paper. Hoyle, incidentally, coined the term Big Bang while rejecting the theory.
The birth of our sun…
Generations of exploded stars of all sizes seeded interstellar space with molecular clouds of raw materials. These clouds contained mostly molecular hydrogen and helium, but also all the other elements made by old stars, for new stars. These were the raw materials for making our solar system.
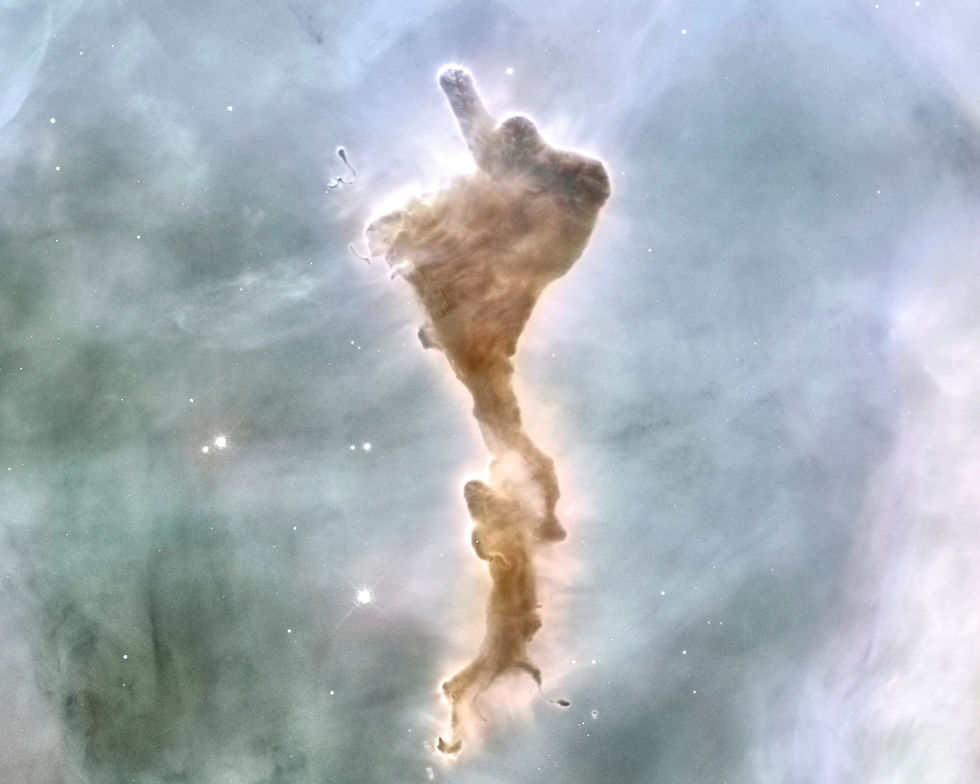
Within the molecular cloud, knots of turbulence formed areas of high concentration, and it is within these knots of dust and gas that the WR star’s wind bubble further compressed matter and triggered our sun to form. First the debris formed a dark, dense, cold protostellar envelope between thousands to tens of thousands of AU in diameter (AU = astronomical unit = the distance from the sun to Earth = 150 million km). Gravity caused the matter at the outer envelope of this dusty region to rain down onto the core of this protostellar cloud perhaps for the first ten-thousand years. By about 100 thousand years, most of the material had collapsed into a whirlpool-like accretion disk which was about 500-1,000 AU in diameter. While matter fell into the accretion disk, some matter was also ejected from the poles of the developing rotating central body, the seed star.
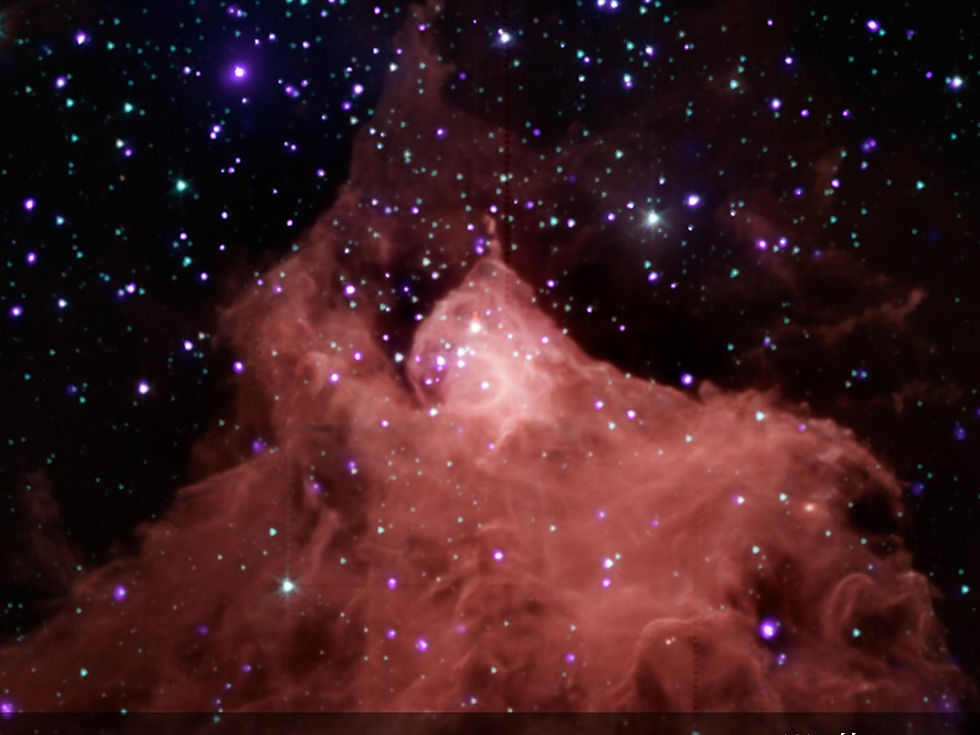
About a million years after the WR star’s triggering event, the sun’s furnace was lit and the new star’s full luminosity drove away most of the remaining gas and dust which had shielded it from view. If we were observing the new-born sun from across the galaxy, it now became optically visible for the first time.
If we could have seen our sun then, we’d see that it retained some of the gas and dust in a ring structure called a protoplanetary disk.
This process took about a million years — a blink of the eye by any temporal measure of the solar system — as if the WR star flipped a switch and the Christmas lights in the stellar nursery flickered on.
But the formation of our solar system around a small, mild, long-lived star was not a given. For example, if our sun had been born in a densely populated stellar nursery with some very big bright supergiants nearby, those massive stars would have burned away the gas and dust around our infant sun before a protoplanetary disk had a chance to form.
From dust to planets…
Before our sun ignited, the dust in the molecular cloud evolved. Elements with high melt temperatures were first to condense out of the gas to grow the nuclei of dust particles. As temperatures dropped, the more volatile materials (including organics and water) deposited later onto those dust nuclei and the particles continued to grew. Collisions between dust particles accelerated their growth.
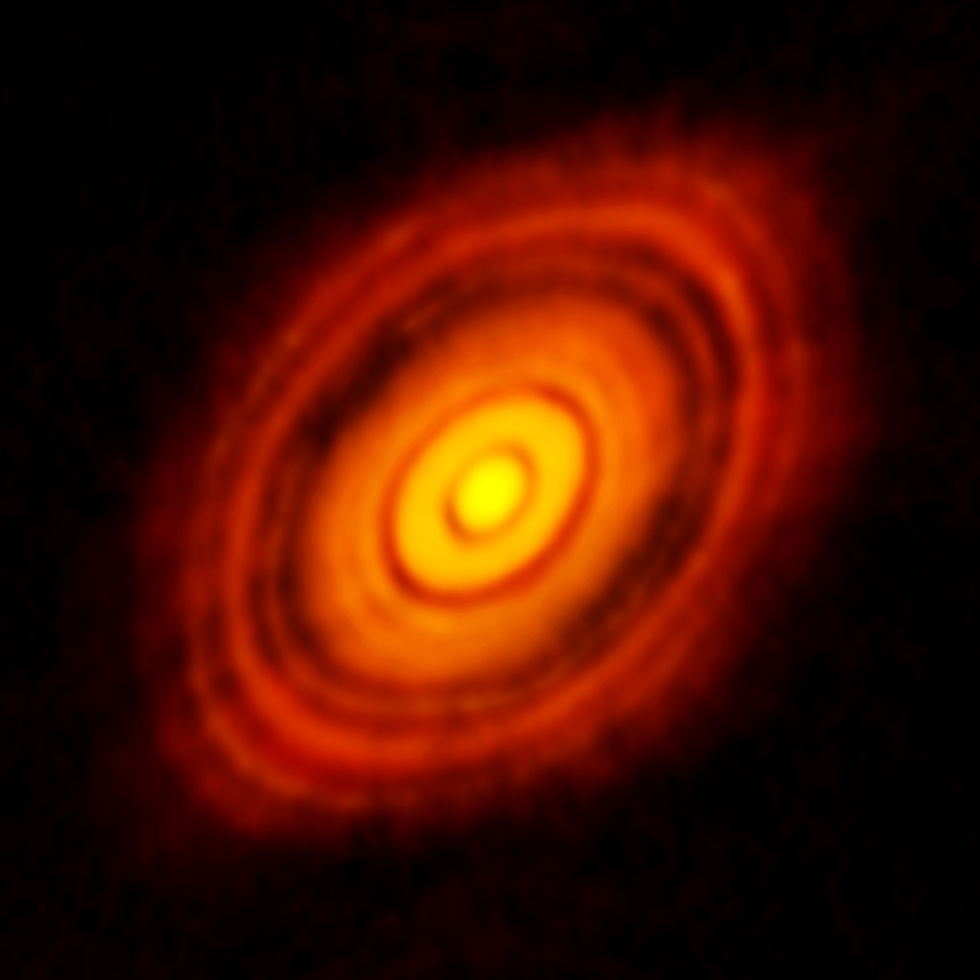
But if the solar wind was too strong, most of the planet-forming materials would have been blown away. If gravity was too strong, most of the materials would have fallen into the sun. Somehow, our system hit a sweet spot, and a stable protoplanetary disk formed, containing all the gas and dust entrained by the newborn sun.
Our sun was lucky to find that balance. The dust slowly aggregated to form planetesimals, but the mechanism of their formation remains a mystery to this day. A number of forces hinder dust grains from evolving into planetesimals (which are defined as bodies between 1–1,000 km in diameter). First, various adhesion forces that help micrometer-sized dust grains glom together to form centimeter-sized pebbles, are insufficient to hold pebbles and larger objects together. Second, gravity is far too weak to reliably form objects at any size below the planetesimal. Third, a variety of conditions within the protoplanetary disk, such as frequent collisions and aerodynamic drag from the gasses in the disk, conspire to tear down what fragile structures were initially built from adhering dust particles. The bottom line is that the weakness of objects from about 1 meter to 1 kilometer in size is a serious obstacle to making planetesimals. Since rocky planets appear common in observable star systems, the obstacle is more in our understanding than in the process of planet formation.
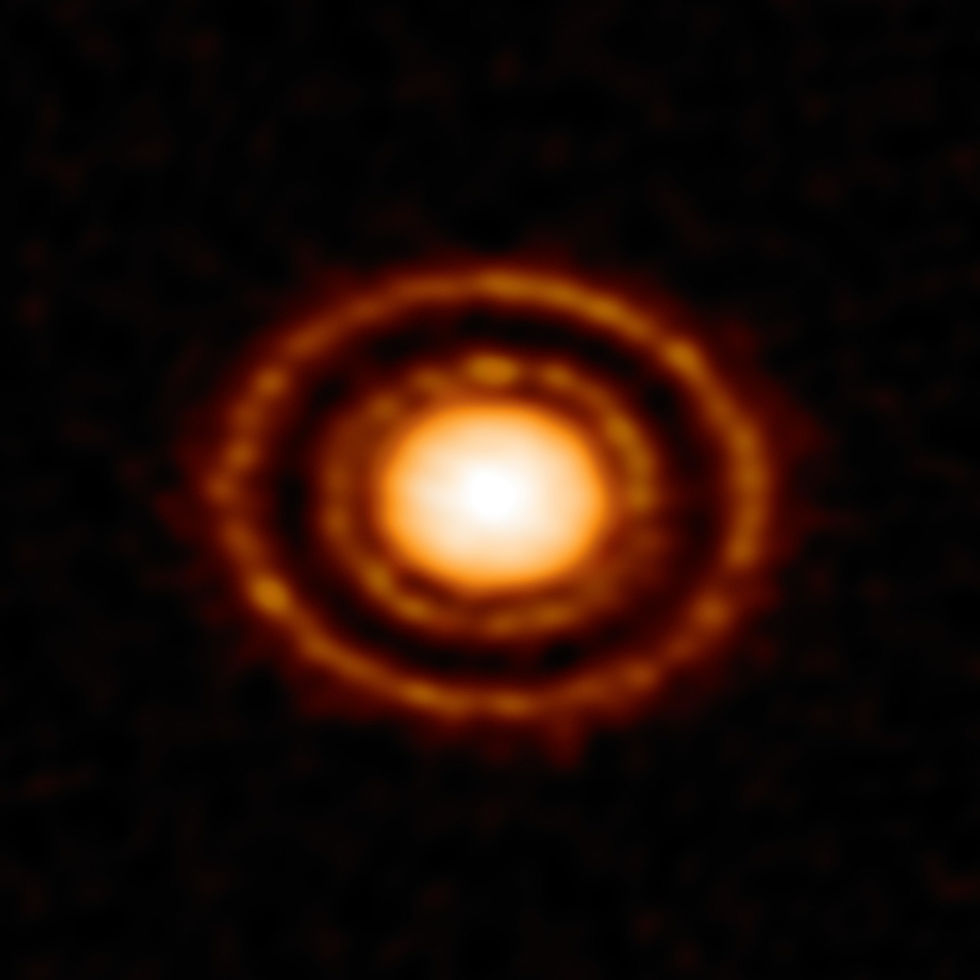
These obstacles were not as relevant for the more distant bodies in the protoplanetary disk. Close to the new-born sun, the blasting heat of radiation burned off volatiles like water. Farther from the sun, water and other volatiles remained on dust particles and larger bodies. A certain distance from the sun (between and beyond the giant planets) the coldness of space and the weakness of solar radiation conspired to form what astronomers call a snow line, analogous to the snow line we see in the mountains. Water ice accumulated, and because it was relatively sticky it helped the larger bodies to form. Imagine how much easier it is to make a softball sized sphere from snow and ice than from sand and gravel. Now think about the fact that the terrestrial planets were made in the very region where the dust and planetesimals must have been blasted dry by the sun. It’s a puzzle, isn’t it?
Nonetheless, somehow, planetesimals, and ultimately dry planets, did form in orbits closest to the sun. We know from observations of other stars similar to ours that protostellar disks, frisbee-shaped clouds of gas and dust surrounding new-born stars, evolved into protoplanetary disks where a stable middle plane allowed dust and gas to aggregate from sub-microscopic sized particles to golf ball sized pebbles. We also know that these early protoplanetary disks evolved rapidly, hollowing out from the inside such that the early sun was surrounded by a dusty donut by 3 million years, and by 6 million years the disk was no longer visible, replaced by a solar system of planetesimals.
The big grow bigger and faster…
Once planetesimals formed in our nascent solar system, growth of the biggest bodies accelerated. The growth of planetesimals followed the pattern we’re all familiar with in corporate America today and in the unfettered days of the Robber Barrons of the late 1800’s… the big grow bigger fastest, everyone else is on the menu as someone’s lunch. Similar to the power law distribution of corporate America, our early solar system was composed of a small number of very large planetesimals, a large number of medium sized bodies, and a huge number of the smallest particles. The gravitational effect of the larger planetesimals began to take over, attracting the smaller bodies and therefore quickly growing and depleting the disk of material. Planetesimals combined to form planetary embryos (or protoplanets) at about the same time that the giant planets formed farther out from the sun.
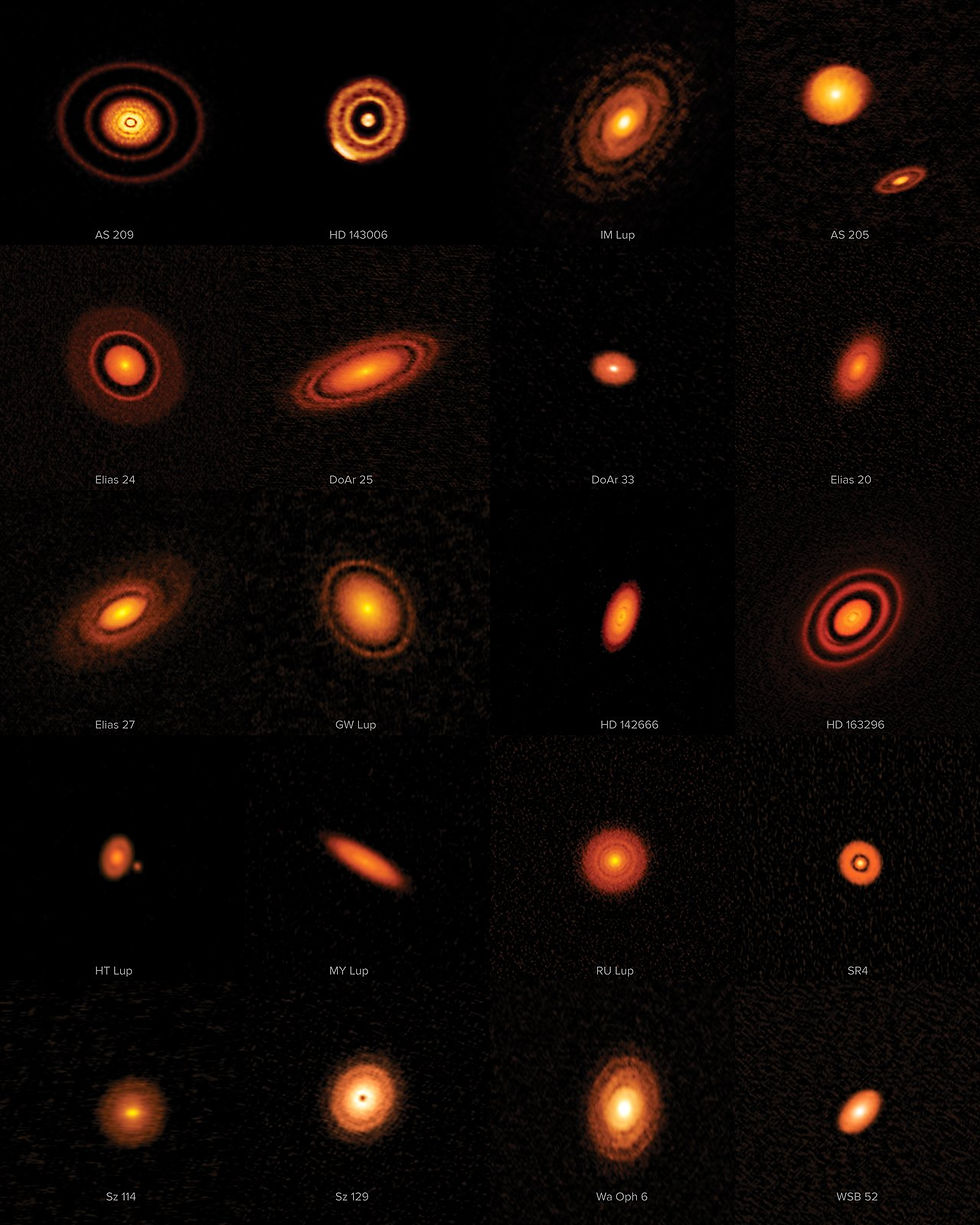
Finally, the planetary embryos crashed among themselves and absorbed the smaller planetesimals to form the terrestrial planets. However, there were a couple interesting twists to this part of the story.
First, we believe that the moon was formed by a Mars-sized protoplanet called Theia crashing into a young proto-Earth. The mechanics of that process are still being worked out, but one theory states that a high-energy impact between two proto-planets vaporized the early Earth. This hot vapor spun quickly, and the vapor created a disk-like shape with the Earth’s heavy core in the middle, connected to a donut-like ring of lighter materials. This vapor disk, called a synestia, is thought to have given rise to a moon with a similar orbital period as our Earth’s rotation. The thorough mixing of the vaporized protoplanets also explains the almost identical chemical compositions of the Earth and its moon. And some believe that our uniquely large moon played an essential role in the development of life on Earth.

Secondly, simulations of the early solar system were unable to generate the terrestrial planets, Mercury, Venus, Earth and Mars, at the correct sizes. Especially Mars. Mars would always come out much larger than the planet we all know and love.
In 2009, Brad Hansen at UCLA’s Department of Physics and Astronomy showed that a particular distribution of material through the disk yielded the correct size of Mars in their simulations.
This inspired a team led by Avi Mandell of NASA to model a novel migration of the giant planets Jupiter and Saturn, which explained Mars’s low mass that we observe today. Previous simulations had all assumed that the giant planet’s orbits were stationary. Avi’s team modeled what happened if the giant planets were allowed to migrate due to the forces from the gas in the disk combined with gravity and other interactions. What this showed is that Jupiter and Saturn migrated inward toward the sun, altered the distribution of the protoplanets which affected the mass of the terrestrial planets, and then migrated back out to their current positions. This maneuver was called the Grand Tack, as the movements of the giant planets was like sailboats tacking back and forth into the wind.
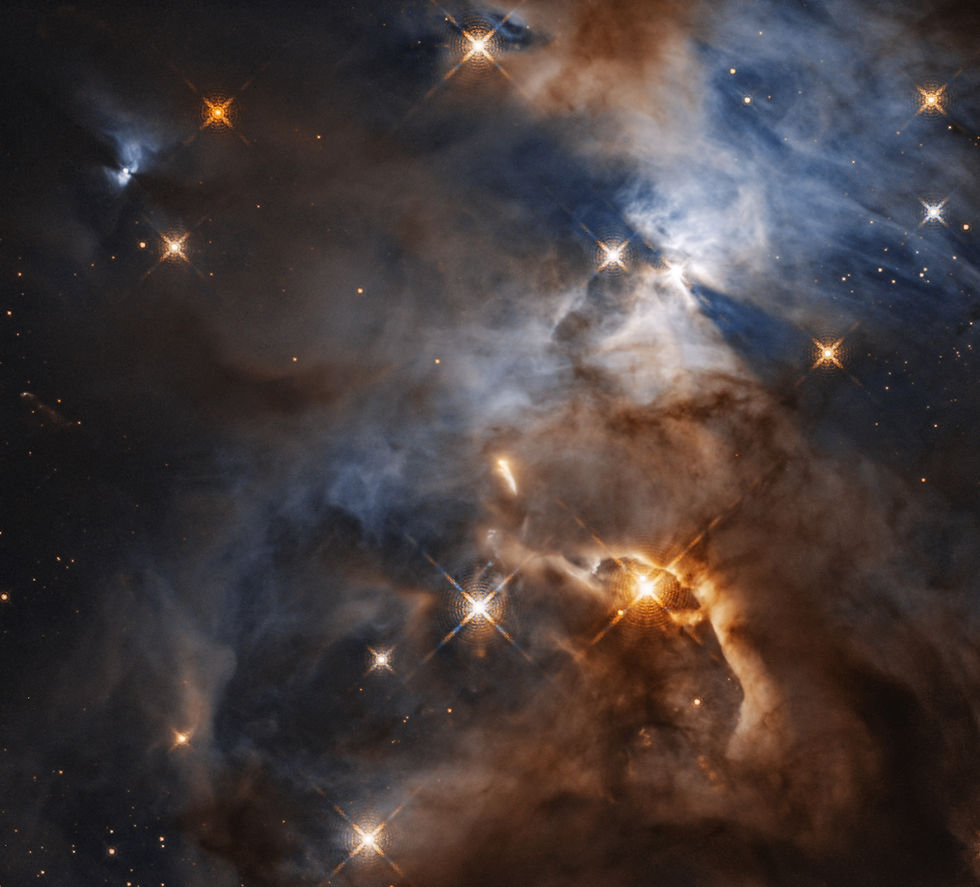
The rapid mass accumulation and Grand Tack migration of Jupiter and Saturn disrupted the orbits of adjacent planetesimals which then bombarded the inner orbits populated by the terrestrial planets. The planetesimals near Jupiter and Saturn were past the snow line and delivered their rich payload of water-ice and organics to the dry planets closest to the sun. We know this based on the isotopes of hydrogen and oxygen in the water here on Earth compared to those isotopes in the sun or in comets or the various types of asteroids. We have also tested the chemistry of meteorites that have landed on Earth, but also directly sampled asteroids and comets in their native habitat.
Along with water, a range of organic compounds were delivered during this bombardment. Nucleobases, the components of DNA and RNA, have been found on carbonaceous meteorites on Earth, which came from asteroids in the outer belts. Amino acids, the building blocks of proteins, have been found on meteorites for decades. Meteorites have also yielded sugars, one of which is ribose, the sugar forming the backbone of RNA. Fatty acids have also been detected on meteorites, and are important since these compounds can form the membranes encapsulating the first protocell.
Water your world and watch it come to life…
Generations of exploded stars of all sizes seeded interstellar space with molecular clouds of raw materials for the next generation of stars and their planets, and ultimately for each of us.
A Wolf-Rayet star’s wind-bubble triggered the formation of our sun from a dormant molecular cloud. A lucky balance of forces ensured that our sun was accompanied by a stable protoplanetary disk that ultimately evolved into our solar system.
The planetesimals and protoplanets evolved rapidly once they reached a critical size. One of the lucky gains in this process was the Earth’s evolution of the moon from a collision with the protoplanet Theia.
The rocky inner planets were initially dry because they formed so close to the sun. By luck, a Grand Tack procession of the giant planets Jupiter and Saturn, first towards and then away from the sun, disrupted neighboring planetesimals which bombarded the terrestrial planets and delivered a payload of water and organic compounds.
On Earth, and perhaps other planets, this delivery of water and organics was essential for the origin of life.
The contribution of extraterrestrial organics to the origin of life is, of course, a huge and unresolved scientific kerfuffle. Many scientists believe that terrestrial prebiotic chemical processes converted simple inorganic gases or other chemicals into precursor organic compounds such as amino acids, nucleotides, fatty acids, and sugars… and then stitched these precursors together into more complex configurations, ultimately into the first living organism.
The hair-pulling and shin-kicking amongst the scientific tribes continues.
What do you think? Which camp are you in?
Thank you!
Once again, you have been patient and followed me to the end of this very, very long story with a most unsatisfactory question at the end.
I will try to follow this with one last article trying to summarize where we are on the origin of life story here on Earth.
Please post this article on your social media or forward by email, snail mail, or sign language to whomever and wherever.
Please subscribe if you haven’t already so I can automatically notify you when I post a new article. And check out all the articles on my blog page here:
Blog | Mysite 1 Blog about science and medicine and history. Science | Hah!scienceduuude.wixsite.com
References…
I apologize for not putting these references in any type of order or formatting them correctly. I mostly wanted them all hyperlinked so you can click directly to the original papers. Hopefully just having the author and title will be sufficient.
コメント